- In-Stock Tumor Cell Lines
- Human Orbital Fibroblasts
- Human Microglia
- Human Pulmonary Alveolar Epithelial Cells
- Human Colonic Fibroblasts
- Human Type II Alveolar Epithelial Cells
- Human Valvular Interstitial Cells
- Human Thyroid Epithelial Cells
- C57BL/6 Mouse Dermal Fibroblasts
- Human Alveolar Macrophages
- Human Dermal Fibroblasts, Adult
- Human Lung Fibroblasts, Adult
- Human Retinal Muller Cells
- Human Articular Chondrocytes
- Human Retinal Pigment Epithelial Cells
- Human Pancreatic Islets of Langerhans Cells
- Human Kidney Podocyte Cells
- Human Renal Proximal Tubule Cells
Synaptic Plasticity
The roles of neurons in higher brain functions such as learning and memory have been well established. Hebb’s law of learning, developed by a Canadian psychologist Donald Hebb, reveals that the material basis of brain memory is synaptic plasticity. Initially, synaptic plasticity refers to the morphological and functional modifications of synaptic connections between neurons, which are mainly manifested in the forms of long term potentiation (LTP) and long term depression (LTD). When two neurons are connected and a nerve impulse occurs, the synaptic transmission between them is enhanced, resulting in more efficient neuronal activation. LTP is the primary form of synaptic plasticity, and both LTP and LTD serve as the biological basis for higher brain functions, such as learning and memory [1, 2].
Astrocyte and Synaptic Plasticity
After the discovery of various functions of neurons in higher brain activities, astrocytes have also been found to be closely related to higher functions of the brain. After Albert Einstein’s death in the 1950s, it was found that the only difference between his brain and that of a normal human was the greater number and size of astrocytes and their processes. Therefore, it was hypothesized that astrocytes must play significant roles in higher functions of the brain. Over the past two decades, there has been a significant advancement in astrocyte research. As the role of astrocytes in higher brain functions has become clearer, their involvement in synaptic plasticity has also been established. The definition of synaptic plasticity has also been extended and developed. Here, we summarize that astrocytes play a crucial role in synaptic plasticity mainly through a few aspects including calcium signaling, release of gliotransmitters, interactions with neurons, and astrocytic plasticity.
Calcium Signaling and the Release of Gliotransmitters
Earlier studies have demonstrated that calcium signaling in astrocytes regulates synaptic plasticity by inducing astrocytic glutamate release which causes an NMDA receptor-dependent increase in miniature postsynaptic currents (PSCs) frequency by acting on extrasynaptic NMDA receptors [3]. In 2004, it was reported that glutamate released from astrocytes could elicit AMPA receptor-mediated spontaneous excitatory PSCs on pyramidal neurons in the CA1 region, thereby regulating synaptic plasticity [4]. In addition, in vivo cholinergic activity evoked by sensory or electrical stimulation elevates the concentration of calcium ions in hippocampal astrocytes, which in turn induces LTP of hippocampal CA3-CA1 synapses by releasing glutamate [5].
In addition to glutamate, astrocytes also release other gliotransmitters after Ca2+ elevations, most prominently D-serine, the co-agonist of NMDA receptor [6, 7] and ATP [8]. It has been demonstrated that the release of D-serine from astrocytes controls NMDAR-dependent plasticity in neighboring excitatory synapses. Moreover, LTP of synaptic transmission also depends on the release of D-serine from astrocytes [6].
Furthermore, ATP released from astrocytes modulates depressive-like behavior in animal models and is very likely to adjust clinical depression in patients. Additionally, a decreased astrocytic release of ATP lead to less stimulation by P2X2Rs at GABAergic interneurons in the medial prefrontal cortex (mPFC), thus reducing GABAergic inhibition and elevating excitability [9]. Astrocytic ATP release is certainly important in astrocyte-neuron reciprocal communications.
Aside from the typical ones discussed above, lactate is also a crucial gliotransmitter especially to postsynaptic neurons. Through glycogenolysis, astrocytes convert glycogen into lactate and release it into the synapses via MCT1 or MCT4 transporters. Then neurons are able to take up them through their MCT2 transporters. Lactate is an additional metabolic energy form provided by astrocytes to facilitate neuronal growth and modulate synaptic plasticity [10].
Influence on Synaptic Transmission through Interactions with Neurons
Besides regulating neuronal activity via the release of gliotransmitters, astrocytes also perform their functions in synaptic transmission by interacting with neurons through their astrocytic receptors located on their extracellular surface. For example, it was pointed out that astrocytic regulation of synapses is mainly based on intracellular Ca2+-dependent processes as well as results from receptor activation, particularly group I metabotropic glutamatergic receptors (mGluRs). Among these, subtype 5 receptors (mGluR5) are the major ones sensing the synaptic release of glutamate at astrocytic processes [11], rather than the low-affinity and rapidly desensitizing AMPAR on neurons [12]. Following their activation through mGluR5 during basal synaptic transmission, astrocytes increase the transmission efficiency in CA1 pyramidal cells via presynaptic adenosine A2A receptors activation, suggesting their direct involvement with neurons in regulation of synaptic transmission [11].
P2X and P2Y receptors are specific receptors that bind to ATP and were determined to be expressed on both of neuronal and astrocytic cell membranes [13]. P2YRs expressed in astrocytes are involved in maintaining neural functions and astrocyte-neuron communications [14]. Stimulation of P2Y1Rs attenuates astrocytic NMDAR currents in PFC, but activation of P2Y4Rs by ATP releases vesicular glutamate from astrocytes, so as to facilitate NMDAR currents [15]. What’s more, the activation of astrocytic P2X7R by ATP results in the release of other gliotransmitters including glutamate and gamma-aminobutyric acid (GABA), subsequently stimulating neighboring neurons and regulating neuronal functions [13, 16, 17].
Moreover, a family of transmembrane proteins called EphrinBs and Eph receptors expressed in the synapses used to be known to regulate synaptic transmission and plasticity [18]. In addition to their presence on CA1 hippocampal neurons, they were also determined to be expressed on hippocampal astrocytes and regulate D-serine synthesis and release [7]. In addition, it was found that astrocytes communicate with neurons through interaction between EphrinA3, a ligand of EphA4 that is found in astrocytes, and EphA4 to modulate LTP [19]. Meanwhile, glutamate transporter 1 (GLT-1/EAAT2) is responsible of regulating the concentration of extracellular glutamate during the late phase of LTP. The interaction between EphrinA3 and EphA4 is also known to be involved in decreasing levels of GLT-1 for proper synapsing to occur [10]. Apparently, the induction of postsynaptic neuronal LTP requires the participation of astrocytes. Figure 1 shows the integrative model proposed for the postsynaptic neuron-astrocyte communication during memory formation [10].
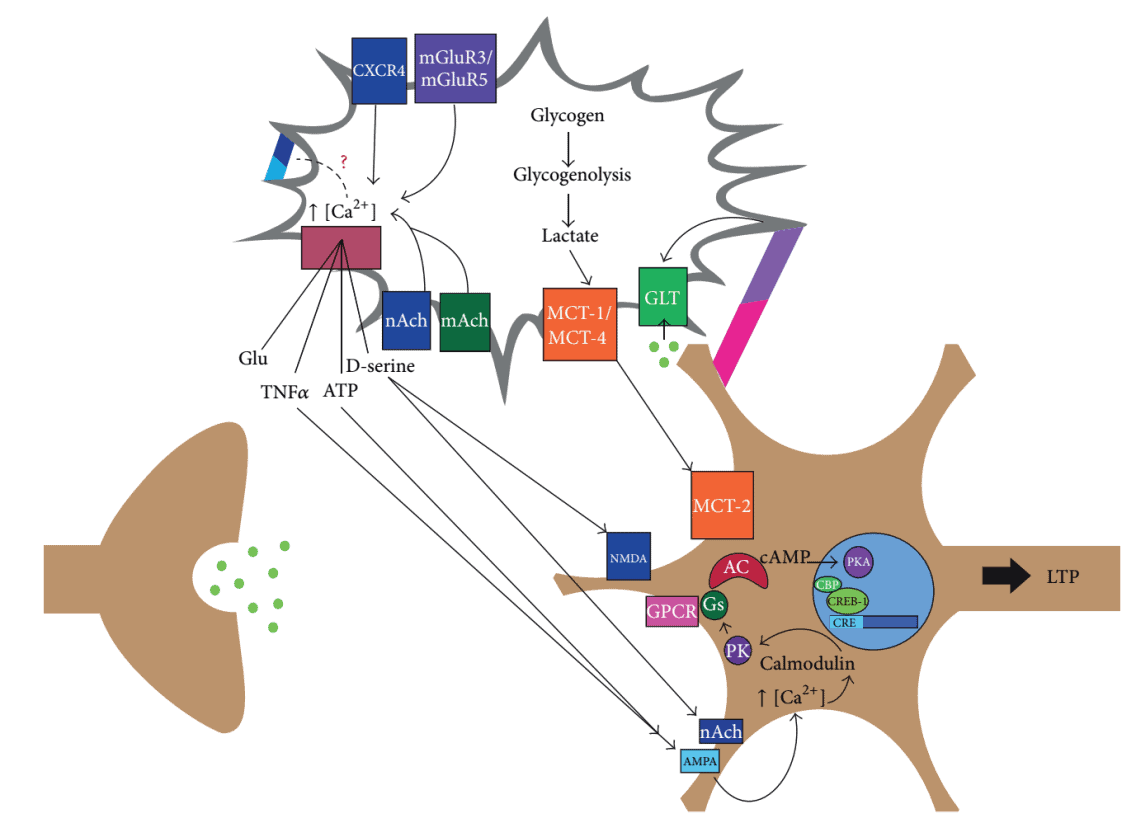
Figure 1. Integrative model of postsynaptic neuron-astrocyte communication during memory formation.
Additionally, astrocytes also perform functions in memory-disruptive effects of intoxication through their receptors. A typical example of astrocyte-neuron interactions is that cannabinoid exposure in vivo activates astrocytic type-1 cannabinoid receptor (CB1R) to induce glutamate release, which further activates NMDAR, triggering AMPAR internalization at CA3-CA1 synapses. These events ultimately lead to the induction of LTD at these synapses, altering the function of hippocampal circuits that likely become unable to process spatial working memory (SWM) [20]. It is worth to mention, the key mechanism involved in this case was largely dependent on the function of astrocytic CB1R rather than on neuronal CB1R, revealing a novel mechanistic views of the critical astrocytic role in learning and memory processes [20].
Astrocytic Plasticity
Astrocytes themselves are malleable and also known to exhibit plasticity, particularly under activation [21]. In rodent neocortical synaptic structures, 30-60 % is tripartite structures formed between astrocytes and neurons. In the hippocampus and somatosensory cortex IV, 60-90 % of the spiny protrusions are enveloped by astrocyte processes. The potential of astrocytic plasticity to control breastfeeding by affecting synapse formation through cellular process migration is one of its recognized examples [22]. Furthermore, “Super Mice” created by chimerizing human astrocytes into the mouse brain have dramatically enhanced their synaptic plasticity, as well as their fast learning and memory abilities [23]. Therefore, astrocytic plasticity directly impacts synaptic plasticity.
In order to better understand different perspectives on astrocyte and its relationship with plasticity, we have summarized the plasticity-related molecules into three categories: astrocytic plasticity (Astroplasticity), astrocytic plasticity in tripartite structure (Perisynaptic Astroplasticity) and neuronal plasticity (Neuroplasticity) in Table 1.
Astroplasticity | Perisynaptic Astroplasticity | Neuroplasticity |
---|---|---|
Ca2+ [5-8, 24] | Ca2+ [3, 25] | Ca2+ [26] |
Glutamate [3, 5, 13, 17] | Glutamate [4, 10, 13, 16, 19, 20, 27] | Glutamate [28] |
D-Serine [7] | D-Serine [6] | NMDAR [3, 6, 7, 15, 20] |
mGluRs [29-31] | mGluR5 [11, 27, 32] | AMPAR [4, 20, 33] |
Glutamine synthetase [34] | Glutamine synthetase [32, 35] | Serine racemase [34] |
Eph receptors [7] | EphrinA3 [10, 19] | Ephrins [18] |
EphrinBs [7] | EphA4 [10, 19] | |
Eph receptors [18] | ||
ATP [9, 13, 17] | ATP [8, 15, 16] | A2A receptor [11] |
Adenosine [10] | A1 receptor [36] | |
P2XRs [14] | P2XRs [13] | P2X2Rs [9] |
P2YRs [14] | P2YRs [13] | P2X7Rs [13] |
P2Y1Rs [15] | P2YRs [13] | |
P2Y4Rs [15] | ||
P2X7Rs [16] | ||
Lactate [10] | MCT2 [10] | |
MCT1 [10] | ||
MCT4 [10] | ||
GLT-1/EAAT2 [37] | GLT-1/EAAT2 [10, 27, 38] | |
GLAST/EAAT1 [39] | GLAST/EAAT1 [27, 38] | |
GABA [40] | GABA [16, 17] | GABA [41] |
GABAA receptor [16] | GABAB receptors [42] | |
GABAB receptor [43, 44] | GABABL receptor [45] | |
CB1R [20] | CB1R [46] | |
GFAP [24, 39, 47-49] | Ezrin [32] | |
NHERF1 [39] | Actin [32] | |
Ezrin [39] | ||
AQP4 [37, 49] | AQP4 [27, 50] | |
AQP5 [51] | Kir4.1 [27, 50, 52] | |
CaM Kinase II [53] | ||
BDNF [54] | ||
Arc [54, 55] | ||
TrkB receptor [54] | ||
Synapsin [56] | ||
Ach [10] | Ach [10, 57] | |
AchR [10, 57] | ||
Map2 [57, 58] | ||
Myosin V [59] |
Conclusion
Astrocyte plays a crucial role in accomplishing neural activities in the brain by regulating both synaptic and non-synaptic plasticity (astrocytic plasticity), as well as working together with neurons through signal exchange. Comprehensive studies of astrocytes and their roles in plasticity are still on-going, the upcoming results will provide us opportunities to better understand the mechanisms underlying higher brain functions from multiple perspectives.
Reference
[1] T.V. Bliss, G.L. Collingridge, A synaptic model of memory long-term potentiation in the hippocampus, Nature 361 (1993) 9.
[2] D.J. Linden, Long-term synaptic depression in the mammalian brain, Neuron 12 (1994) 16.
[3] A. Araque, P.R. Sanzgiri, V. Parpura, P.G. Haydon, Calcium elevation in astrocytes causes an NMDA receptor-dependent increase in the frequency of miniature synaptic currents in cultured hippocampal neurons, Journal of Neuroscience 18 (1998) 8.
[4] T.A. Fiacco, K.D. McCarthy, Intracellular astrocyte calcium waves in situ increase the frequency of spontaneous AMPA receptor currents in CA1 pyramidal neurons, Journal of Neuroscience 24(3) (2004) 722-732.
[5] M. Navarrete, G. Perea, D. Fernandez de Sevilla, M. Gomez-Gonzalo, A. Nunez, E.D. Martin, A. Araque, Astrocytes mediate in vivo cholinergic-induced synaptic plasticity, PLOS Biology 10(2) (2012) e1001259.
[6] C. Henneberger, T. Papouin, S.H. Oliet, D.A. Rusakov, Long-term potentiation depends on release of D-serine from astrocytes, Nature 463(7278) (2010) 232-236.
[7] Z. Zhuang, B. Yang, M.H. Theus, J.T. Sick, J.R. Bethea, T.J. Sick, D.J. Liebl, EphrinBs regulate D-serine synthesis and release in astrocytes, Journal of Neuroscience 30(47) (2010) 16015-16024.
[8] J.-m. Zhang, H.-k. Wang, C.-q. Ye, W. Ge, Y. Chen, Z.-l. Jiang, C.-p. Wu, M.-m. Poo, S. Duan, ATP released by astrocytes mediates glutamatergic activity-dependent heterosynaptic suppression Neuro 40 (2003) 12.
[9] Y.F. Zhao, A. Verkhratsky, Y. Tang, P. Illes, Astrocytes and major depression: The purinergic avenue, Neuropharmacology 220 (2022) 109252.
[10] Y. Ota, A.T. Zanetti, R.M. Hallock, The role of astrocytes in the regulation of synaptic plasticity and memory formation, Neural Plasticity 2013 (2013) 185463.
[11] A. Panatier, J. Vallee, M. Haber, K.K. Murai, J.C. Lacaille, R. Robitaille, Astrocytes are endogenous regulators of basal transmission at central synapses, Cell 146(5) (2011) 785-798.
[12] S.F. Traynelis, L.P. Wollmuth, C.J. McBain, F.S. Menniti, K.M. Vance, K.K. Ogden, K.B. Hansen, H. Yuan, S.J. Myers, R. Dingledine, Glutamate receptor ion channels: structure, regulation, and function, Pharmacological Reviews 62(3) (2010) 405-496.
[13] P. Illes, T.M. Khan, P. Rubini, Neuronal P2X7 Receptors Revisited: Do They Really Exist?, Journal of Neuroscience 37(30) (2017) 7049-7062.
[14] L. Koles, A. Leichsenring, P. Rubini, P. Illes, P2 receptor signaling in neurons and glial cells of the central nervous system, Advances in Pharmacology 61 (2011) 441-493.
[15] K. Wirkner, A. Gunther, M. Weber, S.J. Guzman, T. Krause, J. Fuchs, L. Koles, W. Norenberg, P. Illes, Modulation of NMDA receptor current in layer V pyramidal neurons of the rat prefrontal cortex by P2Y receptor activation, Cereb Cortex 17(3) (2007) 621-631.
[16] M.T. Khan, J. Deussing, Y. Tang, P. Illes, Astrocytic rather than neuronal P2X7 receptors modulate the function of the tri-synaptic network in the rodent hippocampus, Brain Research Bulletin 151 (2019) 164-173.
[17] Y. Zhang, H.Y. Yin, P. Rubini, P. Illes, Y. Tang, ATP indirectly stimulates hippocampal CA1 and CA3 pyramidal neurons via the activation of neighboring P2X7 receptor-bearing astrocytes and NG2 glial cells, respectively, Frontiers in Pharmacology 13 (2022) 944541.
[18] R. Klein, Bidirectional modulation of synaptic functions by Eph/ephrin signaling, Nature Neuroscience 12(1) (2009) 15-20.
[19] A. Filosa, S. Paixao, S.D. Honsek, M.A. Carmona, L. Becker, B. Feddersen, L. Gaitanos, Y. Rudhard, R. Schoepfer, T. Klopstock, K. Kullander, C.R. Rose, E.B. Pasquale, R. Klein, Neuron-glia communication via EphA4/ephrin-A3 modulates LTP through glial glutamate transport, Nature Neuroscience 12(10) (2009) 1285-1292.
[20] J. Han, P. Kesner, M. Metna-Laurent, T. Duan, L. Xu, F. Georges, M. Koehl, D.N. Abrous, J. Mendizabal-Zubiaga, P. Grandes, Q. Liu, G. Bai, W. Wang, L. Xiong, W. Ren, G. Marsicano, X. Zhang, Acute cannabinoids impair working memory through astroglial CB1 receptor modulation of hippocampal LTD, Cell 148(5) (2012) 1039-1050.
[21] C. Yang, S. Rahimpour, A.C. Yu, R.R. Lonser, Z. Zhuang, Regulation and dysregulation of astrocyte activation and implications in tumor formation, Cellular and Molecular Life Sciences 70(22) (2013) 4201-4211.
[22] L.S. Perlmutter, C.D. Tweedle, G.I. Hatton, Neuronal-glial plasticity in the supraoptic dendritic zone dendritic bundling and double synapse formation at parturition, Neuroscience 13 (1984) 11.
[23] X. Han, M. Chen, F. Wang, M. Windrem, S. Wang, S. Shanz, Q. Xu, Nancy A. Oberheim, L. Bekar, S. Betstadt, Alcino J. Silva, T. Takano, Steven A. Goldman, M. Nedergaard, Forebrain Engraftment by Human Glial Progenitor Cells Enhances Synaptic Plasticity and Learning in Adult Mice, Cell Stem Cell 12(3) (2013) 342-353.
[24] K. Gao, C.R. Wang, F. Jiang, A.Y.K. Wong, N. Su, J.H. Jiang, R.C. Chai, G. Vatcher, J. Teng, J. Chen, Y.-W. Jiang, A.C.H. Yu, Traumatic scratch injury in astrocytes triggers calcium influx to activate the JNK/c-Jun/AP-1 pathway and switch on GFAP expression, Glia 61(12) (2013) 2063-2077.
[25] Y. Bernardinelli, J. Randall, E. Janett, I. Nikonenko, S. Konig, E.V. Jones, C.E. Flores, K.K. Murai, C.G. Bochet, A. Holtmaat, D. Muller, Activity-dependent structural plasticity of perisynaptic astrocytic domains promotes excitatory synapse stability, Current Biology 24(15) (2014) 1679-1688.
[26] T.G. Oertner, A. Matus, Calcium regulation of actin dynamics in dendritic spines, Cell Calcium 37(5) (2005) 477-482.
[27] J.P. Heller, D.A. Rusakov, Morphological plasticity of astroglia: Understanding synaptic microenvironment, Glia 63(12) (2015) 2133-2151.
[28] C. Cervetto, A. Amaroli, S. Amato, E. Gatta, A. Diaspro, G. Maura, A. Signore, S. Benedicenti, M. Marcoli, Photons Induce Vesicular Exocytotic Release of Glutamate in a Power-Dependent Way, International Journal of Molecular Sciences 24(13) (2023).
[29] G. Perea, A. Yang, E.S. Boyden, M. Sur, Optogenetic astrocyte activation modulates response selectivity of visual cortex neurons in vivo, Nature Communication 5 (2014) 3262-3273.
[30] R. Martín, R. Bajo-Grañeras, R. Moratalla, G. Perea, A. Araque, Circuit-specific signaling in astrocyte-neuron networks in basal ganglia pathways, Science 349 (2015) 730-734.
[31] Y. Tamaru, S. Nomura, N. Mizuno, R. Shigemoto, Distribution of metabotropic glutamate receptor mGluR3 in the mouse CNS differential location relative to pre- and postsynaptic sites, Neuroscience 106 (2001) 23.
[32] M. Lavialle, G. Aumann, E. Anlauf, F. Prols, M. Arpin, A. Derouiche, Structural plasticity of perisynaptic astrocyte processes involves ezrin and metabotropic glutamate receptors, Proceedings of the National Academy of Sciences 108(31) (2011) 12915-12919.
[33] M. Fischer, S. Kaech, U. Wagner, H. Brinkhaus, A. Matus, Glutamate receptors regulate actin-based plasticity in dendritic spines, Nature Neuroscience 3(9) (2000) 887-894.
[34] Y.F. Wang, M.Y. Sun, Q. Hou, V. Parpura, Hyposmolality differentially and spatiotemporally modulates levels of glutamine synthetase and serine racemase in rat supraoptic nucleus, Glia 61(4) (2013) 529-538.
[35] A. Derouiche, M. Frotscher, Astroglial processes around identified glutamatergic synapses contain glutamine synthetase: evidence for transmitter degradation Brain Research 552 (1991) 5.
[36] E.A. Newman, Glial Cell Inhibition of Neurons by Release of ATP, Journal of Neuroscience 23 (2003) 8.
[37] L. Mogoanta, M. Ciurea, I. Pirici, C. Margaritescu, C. Simionescu, D.A. Ion, D. Pirici, Different dynamics of aquaporin 4 and glutamate transporter-1 distribution in the perineuronal and perivascular compartments during ischemic stroke, Brain Pathology 24(5) (2014) 475-493.
[38] K.P. Lehre, N.C. Danbolt, The number of glutamate transporter subtype molecules at glutamatergic synapses chemical and stereological quantification in young adult rat brain, Journal of Neuroscience 18 (1998) 7.
[39] S.M. Sullivan, A. Lee, S.T. Bjorkman, S.M. Miller, R.K. Sullivan, P. Poronnik, P.B. Colditz, D.V. Pow, Cytoskeletal anchoring of GLAST determines susceptibility to brain damage: an identified role for GFAP, Journal of Biological Chemistry 282(40) (2007) 29414-29423.
[40] Y.F. Wang, M.Y. Sun, Q. Hou, K.A. Hamilton, GABAergic inhibition through synergistic astrocytic neuronal interaction transiently decreases vasopressin neuronal activity during hypoosmotic challenge, European Journal of Neuroscience 37(8) (2013) 1260-1269.
[41] M.E. Soden, J.X. Yee, L.S. Zweifel, Circuit coordination of opposing neuropeptide and neurotransmitter signals, Nature 619 (2023) 332-337.
[42] A.K. Filippov, A. Couve, M.N. Pangalos, F.S. Walsh, D.A. Brown, S.J. Moss, Heteromeric assembly of GABA(B)R1 and GABA(B)R2 receptor subunits inhibits Ca(2+) current in sympathetic neurons Journal of Neuroscience 20 (2000) 2867-2873.
[43] E. Hösli, J. Hösli, Evidence for GABAB-receptors on cultured astrocytes of rat CNS: autoradiographic binding studies Experimental Brain Research 80 (1990) 621-625.
[44] M. Nilsson, P.S. Eriksson, L. Rönnbäck, E. Hansson, GABA induces Ca2+ transients in astrocytes, Neuroscience 54 (1993) 605-614.
[45] K.J. Charles, A.R. Calver, S. Jourdain, M.N. Pangalos, Distribution of a GABAB-like receptor protein in the rat central nervous system, Brain Research 989(2) (2003) 135-146.
[46] M. Kano, T. Ohno-Shosaku, Y. Hashimotodani, M. Uchigashima, M. Watanabe, Endocannabinoid-mediated control of synaptic transmission, Physiological Reviews 89(1) (2009) 309-380.
[47] H. Abraham, A. Somogyvari-Vigh, J.L. Maderdrut, S. Vigh, A. Arimura, Rapidly activated microglial cells in the preoptic area may play a role in the generation of hyperthermia following occlusion of the middle cerebral artery in the rat, Experimental Brain Research 153(1) (2003) 84-91.
[48] Y.F. Wang, V. Parpura, Central Role of Maladapted Astrocytic Plasticity in Ischemic Brain Edema Formation, Frontiers in Cellular Neuroscience 10 (2016) 129-136.
[49] Y.F. Wang, G.I. Hatton, Astrocytic plasticity and patterned oxytocin neuronal activity: dynamic interactions, Journal of Neuroscience 29(6) (2009) 1743-1754.
[50] E.A. Nagelhus, T.M. Mathiisen, O.P. Ottersen, Aquaporin-4 in the central nervous system: cellular and subcellular distribution and coexpression with KIR4.1, Neuroscience 129(4) (2004) 905-913.
[51] R.C. Chai, J.H. Jiang, A.Y. Kwan Wong, F. Jiang, K. Gao, G. Vatcher, A.C. Hoi Yu, AQP5 is differentially regulated in astrocytes during metabolic and traumatic injuries, Glia 61(10) (2013) 1748-1765.
[52] K. Higashi, A. Fujita, A. Inanobe, M. Tanemoto, K. Doi, T. Kubo, Y. Kurachi, An inwardly rectifying K(+) channel, Kir4.1, expressed in astrocytes surrounds synapses and blood vessels in brain, American Journal of Physiology-Cell Physiology 281 (2001) 10.
[53] K. Fukunaga, L. Stoppini, E. Miyamoto, D. Muller, Long-term potentiation is associated with an increased activity of Ca2+/calmodulin-dependent protein kinase II, Journal of Biological Chemistry 268(11) (1993) 7863-7867.
[54] Y. Yin, G.M. Edelman, P.W. Vanderklish, The brain-derived neurotrophic factor enhances synthesis of Arc in synaptoneurosomes, Proceedings of the National Academy of Sciences 99 (2002) 6.
[55] J.F. Guzowski, G.L. Lyford, G.D. Stevenson, F.P. Houston, J.L. McGaugh, P.F. Worley, C.A. Barnes, Inhibition of Activity-Dependent Arc Protein Expression in the Rat Hippocampus Impairs the Maintenance of Long-Term Potentiation and the Consolidation of Long-Term Memory, Journal of Neuroscience 20 (2000) 9.
[56] F. Cesca, P. Baldelli, F. Valtorta, F. Benfenati, The synapsins: key actors of synapse function and plasticity, Progress in Neurobiology 91(4) (2010) 313-348.
[57] N.J. Woolf, The Critical Role of Cholinergic Basal Forebrain Neurons in Morphological Change and Memory Encoding: A Hypothesis, Neurobiology of Learning and Memory 66 (1996) 9.
[58] S. Ladrech, M. Lenoir, J. Ruel, J.L. Puel, Microtubule-associated protein 2 (MAP2) expression during synaptic plasticity in the guinea pig cochlea, Hearing Research 186(1-2) (2003) 85-90.
[59] R. Rudolf, C.M. Bittins, H.H. Gerdes, The role of myosin V in exocytosis and synaptic plasticity, Journal of Neurochemistry 116(2) (2011) 177-191.
Copyright - Unless otherwise stated all contents of this website are AcceGen™ All Rights Reserved – Full details of the use of materials on this site please refer to AcceGen Editorial Policy – Guest Posts are welcome, by submitting a guest post to AcceGen you are agree to the AcceGen Guest Post Agreement – Any concerns please contact [email protected]